Abstract
Conductive metal–organic frameworks (MOFs) are emerging electroactive materials for (opto)electronics; however, it is challenging to achieve MOF-based devices using existing synthesis methods. Here we develop an on-liquid-gallium surface synthesis (OLGSS) strategy under chemical vapour deposition conditions for the controlled growth of two-dimensional conjugated MOF (2D c-MOF) thin films, which gives a tenfold improvement in surface flatness compared with traditionally synthesized c-MOFs. The basis for constructing these flatter surfaces is a layer-by-layer chemical vapour deposition growth mode, which is triggered by the high adhesion energy between gallium and aromatic ligands. We demonstrate the generality of the OLGSS strategy by reproducing flat surfaces for nine different 2D c-MOF films with variable thicknesses (∼2–208 nm). Compared to traditionally synthesized MOF films, the resultant ultrasmooth films enable the formation of high-quality electrical contacts with contact resistance reduced by over 13-fold. Furthermore, due to the efficient interfacial interaction, the prepared van der Waals heterostructure of OLGSS c-MOF and MoS2 shows intriguing photoluminescence enhancement, photoluminescence peak shift and work function modulation. This robust OLGSS method provides the opportunity to develop MOF electronics and shows promise for the construction of multicomponent MOF-based heterostructure materials.
This is a preview of subscription content, access via your institution
Access options
Subscribe to this journal
Receive 12 digital issues and online access to articles
$119.00 per year
only $9.92 per issue
Buy this article
- Purchase on Springer Link
- Instant access to full article PDF
Prices may be subject to local taxes which are calculated during checkout






Similar content being viewed by others
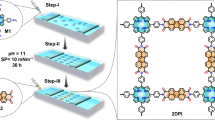
Data availability
The data supporting the findings of the study are available in the paper and its Supplementary Information. Source data are provided with this paper.
References
Talin, A. A. et al. Tunable electrical conductivity in metal–organic framework thin-film devices. Science 343, 66–69 (2014).
Schneider, C. et al. High electrical conductivity and high porosity in a Guest@MOF material: evidence of TCNQ ordering within Cu3BTC2 micropores. Chem. Sci. 9, 7405–7412 (2018).
Liu, J. et al. Photoinduced charge-carrier generation in epitaxial MOF thin films: high efficiency as a result of an indirect electronic band gap? Angew. Chem. Int. Ed. 54, 7441–7445 (2015).
Sun, L., Miyakai, T., Seki, S. & Dincă, M. Mn2(2,5-Disulfhydrylbenzene-1,4-dicarboxylate): a microporous metal–organic framework with infinite (–Mn–S–)∞ chains and high intrinsic charge mobility. J. Am. Chem. Soc. 135, 8185–8188 (2013).
Wang, M., Dong, R. & Feng, X. Two-dimensional conjugated metal–organic frameworks (2Dc-MOFs): chemistry and function for MOFtronics. Chem. Soc. Rev. 50, 2764–2793 (2021).
Liu, J., Chen, Y., Feng, X. & Dong, R. Conductive 2D conjugated metal–organic framework thin films: synthesis and functions for (opto-)electronics. Small Struct. 3, 2100210 (2022).
Sun, L., Campbell, M. G. & Dincă, M. Electrically conductive porous metal–organic frameworks. Angew. Chem. Int. Ed. 55, 3566–3579 (2016).
Xie, L. S., Skorupskii, G. & Dincă, M. Electrically conductive metal–organic frameworks. Chem. Rev. 120, 8536–8580 (2020).
Huang, X. et al. A two-dimensional π–d conjugated coordination polymer with extremely high electrical conductivity and ambipolar transport behaviour. Nat. Commun. 6, 7408 (2015).
Dong, R. et al. A coronene-based semiconducting two-dimensional metal–organic framework with ferromagnetic behavior. Nat. Commun. 9, 2637 (2018).
Chen, S., Dai, J. & Zeng, X. C. Metal-organic Kagome lattices M3(2,3,6,7,10,11-hexaiminotriphenylene)2 (M = Ni and Cu): from semiconducting to metallic by metal substitution. Phys. Chem. Chem. Phys. 17, 5954–5958 (2015).
Dong, R. et al. High-mobility band-like charge transport in a semiconducting two-dimensional metal–organic framework. Nat. Mater. 17, 1027–1032 (2018).
Arora, H. et al. Demonstration of a broadband photodetector based on a two-dimensional metal–organic framework. Adv. Mater. 32, 1907063 (2020).
Sun, L. et al. A microporous and naturally nanostructured thermoelectric metal–organic framework with ultralow thermal conductivity. Joule 1, 168–177 (2017).
Huang, X. et al. Superconductivity in a copper(II)-based coordination polymer with perfect kagome structure. Angew. Chem. Int. Ed. 57, 146–150 (2018).
Wang, Z. F., Su, N. & Liu, F. Prediction of a two-dimensional organic topological insulator. Nano Lett. 13, 2842–2845 (2013).
Liu, J. & Wöll, C. Surface-supported metal–organic framework thin films: fabrication methods, applications, and challenges. Chem. Soc. Rev. 46, 5730–5770 (2017).
Wang, M. et al. Phthalocyanine-based 2D conjugated metal–organic framework nanosheets for high-performance micro-supercapacitors. Adv. Funct. Mater. 30, 2002664 (2020).
Wang, Z. et al. Interfacial synthesis of layer-oriented 2D conjugated metal–organic framework films toward directional charge transport. J. Am. Chem. Soc. 143, 13624–13632 (2021).
Dong, R. et al. Large-area, free-standing, two-dimensional supramolecular polymer single-layer sheets for highly efficient electrocatalytic hydrogen evolution. Angew. Chem. Int. Ed. 54, 12058–12063 (2015).
Wang, L., Sahabudeen, H., Zhang, T. & Dong, R. Liquid-interface-assisted synthesis of covalent–organic and metal–organic two-dimensional crystalline polymers. Npj 2D Mater. Appl. 2, 26 (2018).
Su, P., Tu, M., Ameloot, R. & Li, W. Vapor-phase processing of metal–organic frameworks. Acc. Chem. Res. 55, 186–196 (2022).
Stassen, I. et al. Chemical vapour deposition of zeolitic imidazolate framework thin films. Nat. Mater. 15, 304–310 (2016).
Zeng, M. et al. Bandgap tuning of two-dimensional materials by sphere diameter engineering. Nat. Mater. 19, 528–533 (2020).
Choe, M. et al. Chemical vapor deposition of edge-on oriented 2D conductive metal–organic framework thin films. J. Am. Chem. Soc. 144, 16726–16731 (2022).
Ogle, J. et al. Semiconducting to metallic electronic landscapes in defects-controlled 2D π–d conjugated coordination polymer thin films. Adv. Funct. Mater. 31, 2006920 (2021).
Rubio-Giménez, V. et al. Chemical vapor deposition and high-resolution patterning of a highly conductive two-dimensional coordination polymer film. J. Am. Chem. Soc. 145, 152–159 (2023).
Berro, Y. et al. Atomistic description of phenol, CO and H2O adsorption over crystalline and amorphous silica surfaces for hydrodeoxygenation applications. Appl. Surf. Sci. 494, 721–730 (2019).
Jiang, B., Yang, Z., Liu, X., Liu, Y. & Liao, L. Interface engineering for two-dimensional semiconductor transistors. Nano Today 25, 122–134 (2019).
Fahlman, M. et al. Interfaces in organic electronics. Nat. Rev. Mater. 4, 627–650 (2019).
Rubio-Giménez, V. et al. Bottom-up fabrication of semiconductive metal–organic framework ultrathin films. Adv. Mater. 30, 1704291 (2018).
Song, X. et al. 2D semiconducting metal–organic framework thin films for organic spin valves. Angew. Chem. Int. Ed. 59, 1118–1123 (2020).
Mahringer, A. et al. Oriented thin films of electroactive triphenylene catecholate-based two-dimensional metal organic frameworks. ACS Nano 13, 6711–6719 (2019).
Yao, M. S. et al. Layer-by-layer assembled conductive metal–organic framework nanofilms for room-temperature chemiresistive sensing. Angew. Chem. Int. Ed. 56, 16510–16514 (2017).
Chen, Y. et al. Growth of 2D GaN single crystals on liquid metals. J. Am. Chem. Soc. 140, 16392–16395 (2018).
Liu, J. & Fu, L. Controllable growth of graphene on liquid surfaces. Adv. Mater. 31, 1800690 (2018).
Wundrack, S. et al. Liquid metal intercalation of epitaxial graphene: large-area gallenene layer fabrication through gallium self-propagation at ambient conditions. Phys. Rev. Mater. 5, 024006 (2021).
Lu, W. et al. Controllable sliding transfer of wafer-size graphene. Adv. Sci. 3, 1600006 (2016).
Huang, X. et al. Highly conducting organic–inorganic hybrid copper sulfides CuxC6S6 (x = 4 or 5.5): ligand-based oxidation-induced chemical and electronic structure modulation. Angew. Chem. Int. Ed. 132, 22791–22798 (2020).
Amores, M., Wada, K., Sakaushi, K. & Nishihara, H. Reversible energy storage in layered copper-based coordination polymers: unveiling the influence of the ligand’s functional group on their electrochemical properties. J. Phys. Chem. C 124, 9215–9224 (2020).
Virkar, A. A., Mannsfeld, S., Bao, Z. & Stingelin, N. Organic semiconductor growth and morphology considerations for organic thin-film transistors. Adv. Mater. 22, 3857–3875 (2010).
Chen, Y. et al. Universal growth of ultra-thin III–V semiconductor single crystals. Nat. Commun. 11, 3979 (2020).
Kappera, R. et al. Phase-engineered low-resistance contacts for ultrathin MoS2 transistors. Nat. Mater. 13, 1128–1134 (2014).
Mouri, S., Miyauchi, Y. & Matsuda, K. Tunable photoluminescence of monolayer MoS2 via chemical doping. Nano Lett. 13, 5944–5948 (2013).
Mak, K. F., Lee, C., Hone, J., Shan, J. & Heinz, T. F. Atomically thin MoS2: a new direct-gap semiconductor. Phys. Rev. Lett. 105, 136805 (2010).
Mawlong, L. P. L., Paul, K. K. & Giri, P. K. Direct chemical vapor deposition growth of monolayer MoS2 on TiO2 nanorods and evidence for doping-induced strong photoluminescence enhancement. J. Phys. Chem. C 122, 15017–15025 (2018).
Wang, F. et al. Configuration-dependent electrically tunable van der Waals heterostructures based on MoTe2/MoS2. Adv. Funct. Mater. 26, 5499–5506 (2016).
Kim, G.-S. et al. Schottky barrier height engineering for electrical contacts of multilayered MoS2 transistors with reduction of metal-induced gap states. ACS Nano 12, 6292–6300 (2018).
Zhou, J. et al. A library of atomically thin metal chalcogenides. Nature 556, 355–359 (2018).
Kresse, G. & Furthmüller, J. Efficient iterative schemes for ab initio total-energy calculations using a plane-wave basis set. Phys. Rev. B 54, 11169–11186 (1996).
Kresse, G. & Furthmüller, J. Efficiency of ab-initio total energy calculations for metals and semiconductors using a plane-wave basis set. Comput. Mater. Sci. 6, 15–50 (1996).
Perdew, J. P., Burke, K. & Ernzerhof, M. Generalized gradient approximation made simple. Phys. Rev. Lett. 77, 3865–3868 (1996).
Grimme, S., Antony, J., Ehrlich, S. & Krieg, H. A consistent and accurate ab initio parametrization of density functional dispersion correction (DFT-D) for the 94 elements H–Pu. J. Chem. Phys. 132, 154104 (2010).
Assael, M. J. et al. Reference data for the density and viscosity of liquid cadmium, cobalt, gallium, indium, mercury, silicon, thallium, and zinc. J. Phys. Chem. Ref. Data 41, 033101 (2012).
Ballirano, P., Maras, A., Marchetti, F., Merlino, S. & Perchiazzi, N. Rietveld refinement of chapmanite SbFe2Si2O8OH, a TO dioctahedral kaolinite-like mineral. Powder Diffr. 13, 44–49 (1998).
Jones, L. et al. Smart Align—a new tool for robust non-rigid registration of scanning microscope data. Adv. Struct. Chem. Imaging 1, 8 (2015).
Acknowledgements
This work was financially supported by National Natural Science Foundation of China (22272092), ERC starting grant (FC2DMOF, number 852909), ERC Consolidator grant (T2DCP), SFB-1415 (number 417590517), GRK2861 (number 491865171), EMPIR-20FUN03-COMET, and by the German Science Council, Center for Advancing Electronics Dresden (CFAED). This project has received funding from the European Research Council (ERC) under the European Union’s Horizon 2020 research and innovation programme (ERC grant agreement number 714067, ENERGYMAPS). We acknowledge the European Synchrotron Radiation Facility (ESRF) for provision of synchrotron radiation facilities and we thank O. Konovalov for assistance and support in using beamline ID10. We acknowledge Elettra Sincrotrone Trieste for providing access to its synchrotron radiation facilities and for financial support under the IUS internal project. We thank L. Barba for assistance in using beamline XRD1. We acknowledge Dresden Center for Nanoanalysis (DCN) at TUD. R.D. thanks the Taishan Scholars Program of Shandong Province (tsqn201909047) and the Natural Science Foundation of Shandong Province (ZR2023JQ005). We thank Z. Wang for his help with TEM measurements. J.L. gratefully acknowledges funding from the Alexander von Humboldt Foundation.
Author information
Authors and Affiliations
Contributions
R.D. and X.F. conceived this project. J.L. and Y.C. carried out the CVD growth experiments and the Raman, PL, AFM and SEM measurements, and the device fabrication. X.H. provided the BHT ligand. Y.R., D.B., A.D., J.G. and G.C. conducted the DFT calculations. M.D., F.Z, J.H. and Y.V. performed the XPS and UPS measurements and analysed the spectra. D.P., F.Z., X.L., B.Z. and Z.L. performed the TEM measurements. M.L. contributed to the SEM measurements. M.H. and S.C.B.M. performed the GIWAXS measurements and also contributed to the device measurements. J.L., Y.C., R.D. and X.F. co-wrote the manuscript with contributions from all the authors.
Corresponding authors
Ethics declarations
Competing interests
The authors declare no competing interests.
Peer review
Peer review information
Nature Synthesis thanks Mark Allendorf, Ning Huang, Grigorii Skorupskii and the other, anonymous, reviewer(s) for their contribution to the peer review of this work. Primary Handling Editor: Alison Stoddart, in collaboration with the Nature Synthesis team.
Additional information
Publisher’s note Springer Nature remains neutral with regard to jurisdictional claims in published maps and institutional affiliations.
Supplementary information
Supplementary Information
Supplementary Figs. 1–55 and Tables 1–3.
Source data
Source Data Fig. 3
Statistical source data of film roughness and thickness.
Source Data Fig. 5
Source sata of lateral and vertical devices.
Source Data Fig. 6
Source data of PL, UPS and c-AFM curves.
Rights and permissions
Springer Nature or its licensor (e.g. a society or other partner) holds exclusive rights to this article under a publishing agreement with the author(s) or other rightsholder(s); author self-archiving of the accepted manuscript version of this article is solely governed by the terms of such publishing agreement and applicable law.
About this article
Cite this article
Liu, J., Chen, Y., Huang, X. et al. On-liquid-gallium surface synthesis of ultrasmooth thin films of conductive metal–organic frameworks. Nat. Synth (2024). https://doi.org/10.1038/s44160-024-00513-9
Received:
Accepted:
Published:
DOI: https://doi.org/10.1038/s44160-024-00513-9