Abstract
Metal-support interactions can dramatically affect the properties of nanocomposite materials. Nevertheless, comprehensive studies of the interfaces between metal nanoparticles and oxide supports remain scarce due to challenges in experimental characterization. A significant understanding of the interactions at such interfaces can be obtained by combining state-of-the-art experiments with density functional calculations. In particular, this Perspective illustrates how theory and experiment can be combined to study interfacial charge transfer, the short- or long-range natures of nanoparticle-support interactions and the effects of oxide nanostructuring on the properties of supported metal particles. These studies aid our understanding of the role of metal-oxide interactions in industrially employed nanocomposites and the design of interfaces with unique properties for future applications.
Similar content being viewed by others
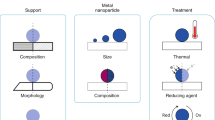
Introduction
Boundaries between substances—interfaces—are ubiquitous in nature and technology. Interfaces between reactive particles and the supports anchoring them can dramatically improve catalytic performance1. Interactions of metal nanoparticles (NPs) with various supports have arguably been discussed for a long time2,3. Many controversies still remain unresolved because even the most modern experimental tools can rarely pinpoint elusive metal-support interactions controlled by several interrelated factors4,5,6,7. However, significant progress has been achieved with computing facilities and quantum-mechanical methods, which have enabled simulations of interfaces in catalytic materials with predictive accuracy. For instance, recent advances in computer codes based on density functional theory (DFT) have enabled reliable calculations of metal-oxide interfaces using realistic models with over one hundred atoms in transition-metal NPs on supports represented by hundreds of atoms (Fig. 1)8. These developments allow DFT simulations to supplement experimental techniques in quantifying and analyzing the effects of supports on the structures and reactivities of deposited metal particles. Understanding these phenomena is the key to preparing tailor-made catalysts and many other complex multicomponent nanomaterials.
Interfaces with surfaces of nonreducible metal oxides
The basics of metal-oxide interactions can be illustrated by considering the effect of the MgO (100) support on the properties of Pd and Pt NPs. In principle, such an effect could be expected to be quite small in the absence of defects in the support, since MgO is a highly ionic and nonreducible oxide. Nevertheless, the MgO (100) support was found to notably affect the stabilities of different shapes and interface configurations of fcc-type metal NPs, while it only slightly altered their interatomic distances and electronic structures8. On average, ca. 0.1 extra electrons were accumulated on metal atoms in direct contact with the support according to a Bader analysis. Figure 2A displays the electron densities induced in Pdn NPs by deposition on MgO (100): in all models, the interface atomic layer revealed the presence of excess electron density, the layer next-nearest to the interface showed minimal depletion of electrons, and basically no charge redistribution was identified far from the atoms at the top of the NP interface.
A Polarization of electron density at the interface of Pd119 and a MgO (100) support rapidly decaying with distance from the support; B calculated adsorption energies in kJ mol-1 for H on Pd127 with respect to ½ H2, (C) O on Pd119 with respect to ½O2, and (D) CO on Pd119 showing how metal-oxide interactions selectively change the reactivities of perimeter sites toward adsorbates; E–G PEEM images showing advancement of the CO deactivation front from the boundaries of 100 μm large Pd particles supported on ZrO2 with increased CO pressure; H CO oxidation activities of Pd particles on Pt and ZrO2 supports depend on the CO pressure and show the beneficial effects of oxide supports on catalyst resistance to CO poisoning. Color coding of atoms in (A–D): Pd – dark and light turquois, Mg – green, O – red. (A is reprinted from Kozlov et al.8, with permission from AIP Publishing; images in (C–H) are adapted with permission from Springer Nature: Suchorski et al.11 copyright 2018).
To what extent does deposition of Pdn and Ptn NPs on MgO (100) modify their reactivity? The difference in the interaction energy of a probe reactant species with a bare and supported NP under scrutiny can serve as an indicator of the effect of interface formation on surface reactivity. To evaluate this effect, DFT calculations were performed on single hydrogen atoms interacting with bare and MgO (100)-supported representative 1.6 nm large particles of Pd127 (Fig. 2B) and Pt127 (not shown)9. Overall, the support changed the binding energies of H to Pd127 and Pt127 NPs by ≤0.07 eV. This effect of the MgO (100) support on the adsorption energy of H was several times smaller than the variation in the H absorption energy inside the NPs, 0.20–0.24 eV, when the surface coverage of H on Pd127 and Pt127 NPs was increased from zero to saturation10.
Notably, the binding of different adsorbates may have different sensitivities to metal-oxide interactions at the perimeter sites on the particle-support interface. For example, Pd/MgO interactions increased the O binding energy by 0.49 eV (from 0.85 to 1.34 eV) at the perimeter sites of the interface, whereas the CO binding energy was increased by only 0.23 eV (from 1.46 to 1.69 eV) at the same sites (Fig. 2C, D)11. Although these values look much higher than the 0.07 eV change in H adsorption energies on the perimeter of the Pd/MgO interface, one should note that these sets of results were obtained by using different NP models. Whereas H binding energies were calculated on Pd127 particles forming an interface with MgO through {100} facets, CO and O were studied on Pd119 particles with {111} facets in contact with the oxide. The interfacial structure or nanoparticle shapes may also affect the binding of an adsorbate to the perimeter sites to a certain extent, and this evaluation requires further study.
A common feature noticed in studies of H, CO or O adsorption on Pd particles supported on MgO were direct effects of nanoparticle-support interactions on the reactivities of metal sites located as far as 0.5 nm from the interface. Despite the apparent short-range nature of metal-oxide interactions, they can have very long-range (>100 μm) implications for catalyst performance. Although characterizing reaction kinetics on such a microscopic scale remains challenging, much can be learned by using photoemission electron microscopy (PEEM), which can reveal the changes in the work function of the explored material induced by the presence of various adsorbates on its surface12,13. For instance, PEEM can show the reaction fronts separating the areas of a CO oxidation catalyst that are covered and poisoned by CO and the areas that are covered by O atoms and remain catalytically active. With a gradual increase in the CO pressure, such reaction fronts were observed to initiate at the particle boundaries and to propagate from there for more than 50 μm to the interiors of particles, gradually changing O-covered areas to areas poisoned by CO11. This mechanism of CO poisoning makes the reaction kinetics highly sensitive to the properties of perimeter sites, where deactivation fronts are initiated. In particular, Pd particles on nonreducible oxide supports show increased energies for O binding on the perimeter sites of metal-oxide interfaces, which hinders competitive CO adsorption on them (Fig. 2E–G). As a result, a doubly higher CO pressure was necessary with micrometer-sized oxide-supported Pd particles to enable the formation of deactivation fronts and propagation across the particle to block the CO oxidation reaction (Fig. 2H). In this way, the effects of metal-support interactions on the reactivities of a small number of sites (<0.001%) on the perimeters of metal-oxide interfaces modify the overall catalytic activity of 100 μm Pd agglomerates. Several oxide supports revealed the beneficial effects of metal-support interactions on the resistance of Pd catalysts to CO poisoning during CO oxidation11. For example, very similar O and CO binding energies were calculated for the perimeter sites of Pd particles supported on MgO and ZrO2. Moreover, Pd particles supported on Al2O3 were also observed to be deactivated at somewhat higher CO pressures than Pd (111), although the required pressure was not as high as that for Pd particles on ZrO2. Obviously, the presence or absence of such beneficial effects depends on the particular combination of a metal and an oxide support.
Interfaces with surfaces of reducible metal oxides: Electron transfer
Electron transfer through the interface is one of the most important components of particle-support interactions. Such charge transfer plays a significant role in the distribution of the electric field and electron polarization in the nanocomposite material and changes the very basics of the electronic structure and the chemical properties of the involved atoms.
The driving force for interfacial electron transfer is the difference between the Fermi levels of the materials forming the interface. The electron transfer also requires the presence of suitable occupied and unoccupied electronic states in the donor and acceptor materials, respectively. Whereas metals have plenty of electronic states at the Fermi level, only some oxide materials will have an electronic structure suitable for interfacial electron transfer. In particular, one could expect that electron transfer would be prominent at the interfaces between metals and reducible metal oxides that allow changes in the oxidation states of metal cations.
Despite the critical importance of interfacial electron transfer on the physical and chemical properties of nanocomposite materials, it is not commonly studied due to the scarcity of suitable experimental techniques. In addition to challenges common to experimental studies of metal-oxide interfaces, characterization of interfacial electron transfer requires extremely sensitive measurements because the process may involve just a few electrons per metal particle. One of the most highly sensitive techniques is resonant photoemission spectroscopy (RPES), which allows one to quantify the extent of metal-oxide charge transfer by measuring concentrations of cations in different charge states on the oxide surface. To achieve that, RPES leverages the strong sensitivity of the resonant condition for the energy of the irradiating phonons with the charge state of the surface cations, thereby enabling the detection of surface cations in a given charge state at concentrations as low as 0.1%14.
For instance, RPES was used to determine with high precision changes in the relative concentrations of surface Ce3+ and Ce4+ cations upon deposition of Pt on ceria support, and this was used to measure the overall magnitude of electron transfer between Pt and ceria15. Moreover, by combining RPES characterization with carefully calibrated X-ray photoelectron spectroscopy measurements to evaluate the overall amount of deposited Pt and scanning tunneling microscopy data to obtain the density of Pt particles on the ceria support, one could derive the number of transferred electrons per Pt atom and per Pt particle (Fig. 3A). In line with the results of DFT calculations, the extent of electron transfer from Pt particles to the ceria support was measured to be ~0.1 electrons per Pt atom for Pt particles measuring 1−1.5 nm in size, i.e., those composed of 30−70 atoms. Deposition of more Pt on ceria resulted in larger Pt particles and saturation of the overall magnitude of metal-oxide electron transfer, which decreased the magnitude of electron transfer per Pt atom. According to DFT data, the saturation of charge transfer and decreased magnitude of charge transfer per Pt atom should also be observed when small Pt particles are deposited on ceria with a high density. Interestingly, the experiments also indicated suppression of electron transfer for very low quantities of deposited Pt. Although this finding was hard to rationalize based on experimental measurements alone, DFT calculations provided a possible mechanistic explanation. Namely, DFT results suggested that the magnitude of electron transfer may have decreased due to interactions between Pt particles and defects on the ceria surface, such as O vacancies, which may serve as nucleation centers for Pt particles at very low Pt coverage16. This study showed how important it is to combine highly sensitive experimental techniques with DFT modeling to obtain a deeper understanding of metal-support charge transfer, which often amounts to just several electrons per metal particle.
A The number of electrons transferred to the support per Pt particle increases with increasing particle size (green squares). The maximum charge per Pt atom is for particles containing 30–70 atoms (yellow circles). B Calculated effect of the number of electrons transferred from Pt8 particles to CeO2 (111) on the adsorption energies Ead of O, CO and H2O species. Color coding of atoms: Pt – dark blue, Ce4+ - beige, Ce3+ - light blue, O – red (darker in the surface layer), C – black. (Adapted with permission from Springer Nature: from Lykhach et al.15 copyright 2016).
Although the extent of metal-oxide electron transfer may be at the detection limits of most experimental techniques, this phenomenon notably affects the properties and catalytic activities of supported metal particles. In view of the experimental challenges, it is more straightforward to study the effects of metal-support electron transfer on the reactivities of supported particles with DFT methods, which allow one to directly control the number of transferred electrons in the simulations. For example, varying the number of electrons transferred from Pt8 clusters to underlying CeO2 (111) from 0 to 3 was calculated to change the energies for binding of CO and H2O molecules on the clusters by almost 0.5 eV (Fig. 3B). Curiously, the energy for binding of O atoms on Pt clusters remained almost the same irrespective of the extent of Pt-ceria electron transfer. Thus, metal-oxide electron transfer may affect the catalytic activity of the supported particles and could also change the selectivity by influencing their interactions with various reaction intermediates to different extents.
Interfaces with nanostructures of reducible metal oxides
Metal oxide supports in catalytic applications commonly do not expose regular extended surfaces. Rather, the supports are present as nanostructures exhibiting a variety of numerous undercoordinated surface sites and irregularities. For the typical reducible metal oxide support CeO2, nanostructuring can result in the formation of surface sites that bind interacting species, e.g., metal atoms, much more strongly than regular surface sites do17,18,19,20. For instance, the square sites of four O atoms exposed by putative lowest-energy structures of stoichiometric (CeO2)n NPs19 were calculated to bind single atoms of late transition metals17,18 even more strongly than these atoms are bound on the surfaces of the corresponding metal particles. Thus, metal particles deposited on ceria can be dispersed, ultimately up to single atoms strongly anchored to such sites. This remarkable behavior (see Fig. 4A) predicted for Pt particles based on DFT simulations was also corroborated experimentally17. Even more interesting, the interplay between ceria-supported Pt particles and cationic single atoms was reversible, and it rapidly adjusted to the oxidative or reductive reaction environment20. These dynamic changes in the structure and electronic state of platinum in the Pt/CeO2 catalyst dramatically affect its reactivity and stability.
A Ceria nanostructures can expose surface sites that very strongly bind single metal atoms and enable dispersion of metal particles as illustrated for Pt9 on Ce40O80. Binding energies of atomic Pt and Pt particles with ceria are shown in italics. Pt - blue, O - red, Ce4+ - beige and Ce3+ - brown. (reprinted from Bruix et al.17 copyright 2014 John Wiley & Sons). B Easier O vacancy formation (oxygen release) in ceria nanoparticles vs. extended ceria systems makes lattice O spillover to Pt particles in Pt/CeO2 composites feasible, as illustrated with Pt8/Ce40O80 models22. C Color coding is the same as that in (A). Charge transfer upon Pt deposition on nanostructured CeO2 at 300 K and O spillover to Pt above 500 K22 (adapted with permission from Springer Nature: from Vayssilov et al.22 copyright 2011).
Another peculiarity of ceria NPs is their much greater propensity to release (and store) oxygen compared to regular surfaces or bulk of ceria. This property is related to the much lower energy required to form oxygen vacancies in nanoparticulate ceria and was rationalized in detail by using DFT calculations more than a decade ago21. At that time, it was predicted that release of lattice O atoms from Ptn/CeO2 NPs with subsequent migration and binding of O to the deposited Ptn species, the so-called reverse spillover of oxygen, was energetically favorable (see Fig. 4B)22. Dedicated RPES experiments demonstrated (see Fig. 4C) that spillover indeed took place as an activated process at moderately elevated temperatures22. This led to a very important conclusion that even under high-vacuum conditions, platinum particles deposited on nanostructured ceria underwent mild partial oxidation by O atoms of the support, resulting in different electronic states and reactivities of such particles compared to those of pristine metallic particles. It should be noted that catalytic Ptn/CeO2 systems can transform in the presence of atmospheric oxygen into oxide-like PtnOm/CeO2 systems with cationic platinum and unique catalytic properties23. In a very recent DFT study24, CeO2-supported PtnOm aggregates were found to be most stable under realistic conditions at stoichiometries close to PtO1.5, whereas the corresponding bare Ptn species tend to be completely oxidized to PtO2 under similar conditions.
Most of the discussion above was focused on the noble metals Pt and Pd. Less noble metals can interact with reducible oxide supports more strongly, resulting in even more significant changes in the catalytic activity. For example, unlike Pt, metals such as Rh do not require nanostructuring of the ceria support to enable reverse O spillover25. Moreover, the interactions of metals such as Ru26 and Cu27 with the ceria support are known to activate the oxide by inducing the formation of O vacancies. In principle, dramatic changes in catalytic activity due to the creation of O vacancies were also observed for Au NPs on ceria28 and titania29 supports during CO oxidation and water-gas shift reactions, among others. Nevertheless, a closer look at the activities of catalysts comprising oxide-supported Au NPs revealed30 that their activities could not be explained solely by O vacancy formation in the support but were also affected by the high intrinsic activities of metal-oxide interfaces in H231 and O232 activation.
Concluding remarks
In summary, a growing understanding of the processes operating on metal-oxide interfaces shows their critical importance in determining the properties of many nanocomposite materials. Metal-oxide interactions may profoundly affect both the geometric and electronic structures of supported metal NPs as well as their reactivities. However, the effect of the support on particle properties may vary greatly depending on the property under consideration as well as the distance to the metal-oxide interface. Some properties, such as interatomic distances or polarization of electron density in the nanoparticle, seem to be altered only in the immediate vicinity of the interface33. In contrast, the effects of support on the shapes and charge states of NPs naturally extend to the entire particles.
The effects of metal-oxide interactions on the reactivities of NPs are found to be more complex. The interactions between metal particles and some adsorbates seem to be essentially insensitive to the nanoparticle-support interactions, whereas binding energies to other species can change dramatically, by up to 0.5 eV, in the presence of oxide support. This indicates that nanoparticle-support interactions may govern the activities of nanocomposite materials in catalytic applications as well as their selectivities. Although oxide supports noticeably affect the binding energies of various adsorbates and reaction intermediates only on the perimeter sites of the nanoparticle-support interfaces, such sites were demonstrated to have far-reaching implications for catalytic activity. Moreover, metal-oxide interactions often change the catalytic activities and other properties of oxide supports by facilitating O vacancy formation on their surfaces and reverse spillover of O atoms from the oxide to metal NPs.
One of the most exciting components of nanoparticle-support interactions is charge transfer through the metal-oxide interface. Available studies suggest that this electron transfer is facilitated by the reducible nature of the oxide support and strongly depends on the presence of defects in the oxide and its nanostructuring. Although interfacial electron transfer appears to achieve the highest magnitude per atom for ~1.5 nm particles, which may cause the accumulation of only several electrons, it may significantly affect the electronic structures and reactivities of the NPs.
Current studies of nanoparticle-support interactions are limited by the insufficient sensitivity or resolution of customary characterization techniques and challenges in applying them to materials with complex morphologies. As a result, the most impressive advances in this area have been achieved with combinations of sophisticated experimental techniques and density functional simulations, which provide the necessary atomic-level insight into the electronic structures and reactivities of the nanocomposite materials. Although atomic-level studies of metal-oxide interfaces have gained some momentum, future progress will depend on development of experimental techniques and computational approaches to treat systems of such complexity seamlessly.
A better understanding of the unique properties of metal-oxide interfaces in nanocomposite materials will facilitate engineering for a given application. In particular, one could expect nanocomposites enhanced by tailored metal-oxide interactions to lead to breakthroughs in catalysis, plasmonics and related applications.
References
Carrettin, S., Concepción, P., Corma, A., López Nieto, J. M. & Puntes, V. F. Nanocrystalline CeO2 increases the activity of Au for CO oxidation by two orders of magnitude. Angew. Chem. Int. Ed. 43, 2538–2540 (2004).
Tauster, S. J., Fung, S. C., Baker, R. T. K. & Horsley, J. A. Strong interactions in supported metal catalysts. Science 211, 1121–1125 (1981).
Campbell, C. T. Catalyst-support interactions: Electronic perturbations. Nat. Chem. 4, 597–598 (2012).
Ahmadi, M., Mistry, H. & Roldan Cuenya, B. Tailoring the catalytic properties of metal nanoparticles via support interactions. J. Phys. Chem. Lett. 7, 3519–3533 (2016).
Ro, I., Resasco, J. & Christopher, P. Approaches for understanding and controlling interfacial effects in oxide-supported metal catalysts. ACS Catal. 8, 7368–7387 (2018).
Ruiz Puigdollers, A., Schlexer, P., Tosoni, S. & Pacchioni, G. Increasing oxygen reducibility: The role of metal/oxide interfaces in the formation of oxygen vacancies. ACS Catal. 7, 6493–6513 (2017).
Pacchioni, G. & Freund, H.-J. Controlling the charge state of supported nanoparticles in catalysis: lessons from model systems. Chem. Soc. Rev. 47, 8474–8502 (2018).
Kozlov, S. M., Aleksandrov, H. A., Goniakowski, J. & Neyman, K. M. Effect of MgO(100) support on structure and properties of Pd and Pt nanoparticles with 49-155 atoms. J. Chem. Phys. 139, 084701 (2013).
Kozlov, S. M., Aleksandrov, H. A. & Neyman, K. M. Adsorbed and subsurface absorbed hydrogen atoms on bare and MgO(100)-supported Pd and Pt nanoparticles. J. Phys. Chem. C. 118, 15242–15250 (2014).
Kozlov, S. M., Aleksandrov, H. A. & Neyman, K. M. Energetic stability of absorbed H in Pd and Pt nanoparticles in a more realistic environment. J. Phys. Chem. C. 119, 5180–5186 (2015).
Suchorski, Y. et al. The role of metal/oxide interfaces for long-range metal particle activation during CO oxidation. Nat. Mater. 17, 519–522 (2018).
Suchorski, Y. & Rupprechter, G. Local reaction kinetics by imaging. Surf. Sci. 643, 52–58 (2016).
Suchorski, Y. et al. Resolving multifrequential oscillations and nanoscale interfacet communication in single-particle catalysis. Science 372, 1314–1318 (2021).
Matolín, V. et al. A resonant photoelectron spectroscopy study of Sn(Ox) doped CeO2 catalysts. Surf. Interfac. Anal. 40, 225–230 (2008).
Lykhach, Y. et al. Counting electrons on supported nanoparticles. Nat. Mater. 15, 284–288 (2016).
Bruix, A. et al. Effects of deposited Pt particles on the reducibility of CeO2(111). Phys. Chem. Chem. Phys. 13, 11384–11392 (2011).
Bruix, A. et al. Maximum noble-metal efficiency in catalytic materials: atomically dispersed surface platinum. Angew. Chem. Int. Ed. 53, 10525–10530 (2014).
Figueroba, A., Kovács, G., Bruix, A. & Neyman, K. M. Towards stable single-atom catalysts: strong binding of atomically dispersed transition metals on the surface of nanostructured ceria. Catal. Sci. Technol. 6, 6806–6813 (2016).
Bruix, A. & Neyman, K. M. Modeling ceria-based nanomaterials for catalysis and related applications. Catal. Lett. 146, 2053–2080 (2016).
Lykhach, Y. et al. Oxide-based nanomaterials for fuel cell catalysis: the interplay between supported Pt atoms and particles. Catal. Sci. Technol. 7, 4315–4345 (2017).
Migani, A., Vayssilov, G. N., Bromley, S. T., Illas, F. & Neyman, K. M. Greatly facilitated oxygen vacancy formation in ceria nanocrystallites. Chem. Commun. 46, 5936–5938 (2010).
Vayssilov, G. N. et al. Support nanostructure boosts oxygen transfer to catalytically active platinum nanoparticles. Nat. Mater. 10, 310–315 (2011).
Wang, H. et al. Surpassing the single-atom catalytic activity limit through paired Pt-O-Pt ensemble built from isolated Pt1 atoms. Nat. Commun. 10, 3808 (2019).
Quinlivan Domínguez, J. E., Neyman, K. & Bruix, A. Stability of oxidized states of ceria-supported PtOx particles under a wide range of gas-phase conditions. ChemRxiv Cambridge:Cambridge Open Engage; 2021 https://doi.org/10.33774/chemrxiv-2021-rn4p1.
Yentekakisa, I. V. et al. Effect of support oxygen storage capacity on the catalytic performance of Rh nanoparticles for CO2 reforming of methane. Appl. Catal. B: Environ. 243, 490–501 (2019).
Wang, Z. et al. Ru/CeO2 catalyst with optimized CeO2 support morphology and surface facets for propane combustion. Environ. Sci. Technol. 53, 5349–5358 (2019).
Chen, A. et al. Structure of the catalytically active copper–ceria interfacial perimeter. Nat. Catal. 2, 334–341 (2019).
Ha, H., Yoon, S., An, K. & Kim, H. Y. Catalytic CO oxidation over Au nanoparticles supported on CeO2 nanocrystals: effect of the Au–CeO2 interface. ACS Catal. 8, 11491–11501 (2018).
Liu, N. et al. Auδ−–Ov–Ti3+ interfacial site: catalytic active center toward low-temperature water gas shift reaction. ACS Catal. 9, 2707–2717 (2019).
Ishida, T., Murayama, T., Taketoshi, A. & Haruta, M. Importance of size and contact structure of gold nanoparticles for the genesis of unique catalytic processes. Chem. Rev. 120, 464–525 (2020).
Whittaker, T. et al. H2 Oxidation over supported Au nanoparticle catalysts: evidence for heterolytic H2 activation at the metal–support interface. J. Am. Chem. Soc. 140, 16469–16487 (2018).
Huang, J. et al. Manipulating atomic structures at the Au/TiO2 interface for O2 activation. J. Am. Chem. Soc. 142, 6456–6460 (2020).
Kozlov, S. M. & Neyman, K. M. Effects of electron transfer in model catalyst composed of Pt nanoparticles on CeO2(111) surface. J. Catal. 344, 507–514 (2016).
Acknowledgements
The work of K.M.N. was partly funded by the Spanish Government MCIN/AEI/10.13039/501100011033 via grants PGC2018-093863-B-C22 and MDM-2017-0767 as well as by the grant 2017SGR13 from the Generalitat de Catalunya. S.M.K. acknowledges funding from the National Research Foundation under the NRF Fellowship NRFF13-2021-0126. Computer resources provided by the Red Española de Supercomputación are gratefully acknowledged.
Author information
Authors and Affiliations
Contributions
All authors wrote the paper.
Corresponding authors
Ethics declarations
Conflict of interest
The authors declare no competing interests.
Additional information
Publisher’s note Springer Nature remains neutral with regard to jurisdictional claims in published maps and institutional affiliations.
Rights and permissions
Open Access This article is licensed under a Creative Commons Attribution 4.0 International License, which permits use, sharing, adaptation, distribution and reproduction in any medium or format, as long as you give appropriate credit to the original author(s) and the source, provide a link to the Creative Commons license, and indicate if changes were made. The images or other third party material in this article are included in the article’s Creative Commons license, unless indicated otherwise in a credit line to the material. If material is not included in the article’s Creative Commons license and your intended use is not permitted by statutory regulation or exceeds the permitted use, you will need to obtain permission directly from the copyright holder. To view a copy of this license, visit http://creativecommons.org/licenses/by/4.0/.
About this article
Cite this article
Neyman, K.M., Kozlov, S.M. Quantifying interactions on interfaces between metal particles and oxide supports in catalytic nanomaterials. NPG Asia Mater 14, 59 (2022). https://doi.org/10.1038/s41427-022-00405-4
Received:
Revised:
Accepted:
Published:
DOI: https://doi.org/10.1038/s41427-022-00405-4