« Prev Next »
Previous Section: What Is Ocean Productivity?
What Controls Ocean Productivity on Long Time Scales?
Reconstructions of ocean productivity using sediment records typically involve the accumulation of biogenic matter (organics or mineral hard parts) in the sediment; therefore, these studies speak to export production rather than NPP. However, if export production is reconstructed, it is at least a fair assumption that NPP would have changed in the same direction. Moreover, as described below, the export of organic matter out of the surface ocean can have broad biogeochemical and climate implications, so reconstructing export production is valuable in itself. One of the greatest challenges for reconstructing ocean productivity is the potential for changes in the fraction of export production that reaches the seabed and is preserved into the sediments, which could be misinterpreted as changes in productivity. The development and improvement of such reconstructions is an active area of research.
Circulation
Nutrients
Approaches are lacking to directly reconstruct the past reservoir sizes of different ocean nutrients. Nevertheless, a framework for considering the potential for such changes has been developed, and this is our focus below.
Phosphorus
Nitrogen
The input/output budget of ocean N is largely biologically driven. Biologically available (or "fixed") N is brought into the ocean mostly by oceanic N "fixers," a set of cyanobacterial phytoplankton that cleave the strong triple bond between the N atoms of N2 to produce ammonium (NH4+) and then organic N. Once fixed, the N is incorporated into the global ocean N cycle, which is dominated by the large reservoir of nitrate (NO3-) stored in deep water. The upwelling or mixing of this nitrate into the euphotic zone (which along with the slower processes of N fixation and atmospheric N deposition represents the "new" N supply to the euphotic zone) drives the export production across the global ocean (Figure 1). Fixed N is dominantly lost from the ocean by "denitrification," the use of nitrate as an oxidant to decompose organic matter by bacteria that grow in low-oxygen environments. Just as the ocean's fixed N is derived from the huge atmospheric reservoir of N2 by N fixation, denitrification converts the fixed N back to N2. A second widespread reaction removing fixed N in low-oxygen settings is known as "anammox" (for anaerobic ammonium oxidation), which combines ammonium with nitrite (NO2-) to produce N2 (Dalsgaard et al. 2005). The quantitative importance of this process is not yet clear.
Recent decades have seen a number of hypotheses regarding past ocean productivity centered around N reservoir changes. During recent ice ages, there is strong evidence that denitrification slowed (Altabet et al. 1995, Christensen 1994, Ganeshram et al. 1995). It has been proposed that this increased the size of the ocean N reservoir, leading to higher ocean productivity and explaining the observed drop in atmospheric CO2 during ice ages. Since the residence time of N in the ocean is ~3 thousand years (Brandes & Devol 2002) — more than 5-fold shorter than that of P-N could have changed substantially across glacial-to-interglacial transitions and over longer time scales in Earth history. However, a potent argument involving P has been made against such changes in the ocean N budget, as described below.
Dissolved N (dominantly nitrate) is tightly correlated with dissolved P (phosphate, PO43-) in open ocean waters, with an N:P ratio of 16:1 (Figure 6). As this is also the N:P observed in plankton, Redfield (1958) explained the dissolved nitrate-to-phosphate correlation as the result of internal nutrient cycling in the ocean, with N and P consumed from water in a 16:1 ratio by phytoplankton growth and then put back into solution with the same ratio upon "remineralization" (decomposition by heterotrophs) of marine organic matter (Figure 6, green dashed lines). Biological assays tend to indicate that N limits oceanic phytoplankton more often than does phosphorus (Kilham & Hecky 1988), consistent with the observation that there is a positive x-intercept in Figure 6 (nitrate tends to be depleted "before" phosphate).
Assuming that the N:P ratio of most phytoplankton is conserved (i.e., cannot vary so as to compensate for changes in the N:P of the nutrient supply from deep water), then a negative feedback develops between the N:P of the ocean and the rate of N fixation. This feedback has been been proposed to stabilize the ocean N budget as follows (Figure 6). Denitrification removes nitrate from the ocean interior. This leads waters upwelled into the surface to have a deficit in N relative to the N and P demands of phytoplankton growth (or, more precisely, the N and P supply required to balance the N and P exported in sinking organic matter). This leads to N depleted surface waters with "excess P," conditions under which N fixers are surmised to compete successfully. The N fixers then fix the N that compensates for the N deficit in upwelled waters and that balances the N loss due to denitrification \. This feedback has been recognized in lake studies, in which the addition of excess P caused blooms of cyanobacteria, which fixed N and thus shifted the lake back toward its pre-amendment N:P ratio (Schindler 1977), and apparent spatial coupling between denitrification and N fixation support its applicability to the ocean (Deutsch et al. 2007). If the negative feedback is strong in the ocean and the N:P of sinking organic matter is conserved through time, then the ocean N:P cannot stray far from the modern 16:1 ratio, and the P reservoir determines the nutrient reservoir sizes and their effect on ocean productivity (Tyrrell 1999).
In this context, at the end of the last ice age, the increase in denitrification would have lowered the ocean's N reservoir and reduced the ocean's N:P ratio. This appears to have led to an increase in the rate of N fixation (Ren et al. 2009), which worked to stabilize the N reservoir and bring the ocean N budget to a new (interglacial) steady state (Deutsch et al. 2004). This feedback has also been inferred for other events in Earth history, such as the "ocean anoxic events" of the Cretaceous Period, when an acceleration in the denitrification rate apparently led to a compensatory increase in N fixation rate (Rau et al. 1987).
The coupling between N and P in the ocean might be weakened by variation in the N:P stoichiometry of phytoplankton. In recent years, researchers have begun to investigate apparent particulate and dissolved N:P deviations from the modern oceanic mean of 16:1 (Arrigo et al. 1999, Weber & Deutsch 2010). Of particular importance is the question of whether the N:P of organic matter exported out of the surface ocean could vary on a global basis over time. In addition, N fixation requires two metals — iron and molybdenum — that could potentially limit the rate of this process and thus interfere with the N fixation feedback. It has been argued that the scarcity of iron in the modern ocean (see below) contributes to the widespread tendency toward N deficit in the global ocean by suppressing N fixation rates (Falkowski 1997). More dramatically, it has been hypothesized that the long spell of slow evolution in life from 2.0 to 0.6 billion years ago was due to molybdenum limitation of N fixers, which slowed ocean productivity, organic carbon burial, and the build-up of oxygen in the atmosphere (Anbar & Knoll 2002).
Iron
Iron is required for many phytoplankton functions, perhaps most importantly in the electron transport chain of photosynthesis. However, iron was not suspected as a limiting nutrient until the advent of trace metal clean techniques allowed for uncontaminated iron addition experiments and demonstrated that iron has nutrient-like structure in the ocean, with extreme depletion in the surface waters of many regions, especially in polar surface waters with high concentrations of the "major nutrients," N and P (Martin et al. 1989) (Figure 4c and d). In regions with high surface concentrations of the major nutrients, iron addition experiments on diverse scales have consistently yielded large enhancements of NPP, increases in biomass, and changes in phytoplankton assemblage; in several cases, an increase in export production has also been observed (Boyd et al. 2007).
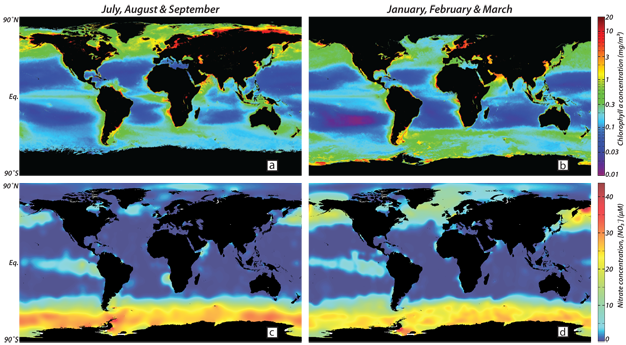

Iron enters the ocean mostly through dust deposition on the ocean surface, although ocean margins and hydrothermal vents are also substantial sources (Figure 5). The nutrient-like depth structure of iron indicates that it is consumed in surface water by phytoplankton and then put back into solution when sinking organic matter is remineralized at depth (Martin et al. 1989). Iron is also known to have an active internal cycle in the euphotic zone, involving both biological processes and reactions with light (Barbeau et al. 2001, Morel et al. 1991). However — unlike CO2, N, P, or Si — iron in the ocean water column precipitates and is scavenged by settling particles that transport it to the seabed, thus removing it from the ocean (Boyd & Ellwood 2010) (Figure 5). As a result, in comparison to the major nutrients, iron is cycled fewer times within the ocean between the times of its input and its removal (Johnson et al. 1997). This difference derives fundamentally from the high O2 of the modern atmosphere and ocean, which ensures that iron is largely present in the low solubility oxidation state of +III. The oxidized nature of the global environment is itself a consequence of past photosynthesis followed by organic carbon burial. Thus, the scarcity of iron in the modern ocean is an example of the ability of Earth's biosphere to affect its own fertility.
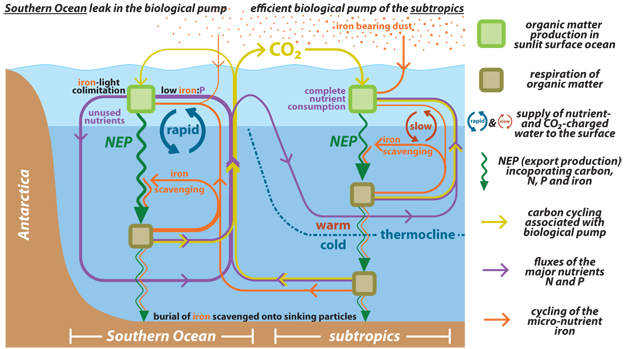

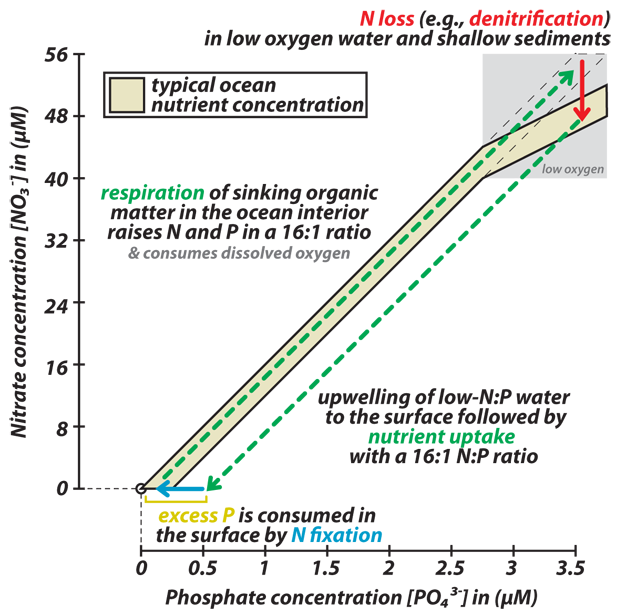

The lower cycling-to-throughput ratio for iron in the ocean helps to explain its tendency to limit productivity in polar waters and in upwellings. Because iron is constantly being scavenged out of ocean water, deep water has a lower iron-to-major nutrient ratio than phytoplankton require. When deep water is brought to the surface, phytoplankton thus run out of iron first, leaving a large fraction of the major nutrients unused (Figure 5, left side). The iron supply from dust and other shallow sources can augment the iron supply in these settings, mitigating but not erasing the inherent iron deficit. In contrast, in the permanently stratified tropical and subtropical ocean where the input of deep water to the surface is very slow, the dust flux from above typically compensates for any iron deficit in the nutrients supplied from below, such that the total iron supply is adequate for the phytoplankton to completely consume the slow supply of major nutrient from below (Figure 5, right side). Thus, these low latitude regions are largely limited by the major nutrients rather than iron.
Because the deep ocean is ventilated largely by the polar ocean regions, the incomplete consumption of nutrients in these regions introduces substantial inefficiency to the global ocean's biological pump (Sarmiento & Toggweiler 1984) (Figure 5, left side). Fertilization with iron represents a potential mechanism for reducing this inefficiency and thus lowering atmospheric CO2. Indeed, John Martin hypothesized that the lower CO2 concentrations observed during ice ages were driven by increased dust flux to the Southern Ocean, which fertilized the region with iron (Martin 1990). This process alone cannot explain all of the data from the ice age Southern Ocean; for example, the apparent ice age decrease in productivity in Antarctic waters requires an alternative or additional change, such as reduced upwelling (Francois et al. 1997, Sigman & Boyle 2000). Nevertheless, iron fertilization does appear to have been important in the ice age Southern Ocean, most clearly the Subantarctic Zone under the westerly wind belt (Kohfeld et al. 2005), and it probably played a part in lowering atmospheric CO2 during ice ages (Watson et al. 2000, Martinez-Garcia et al. 2011).
Silicon
Silicon — as Si(OH)4 — is required by a number of phytoplankton and zooplankton groups for the construction of opal hard parts, all of which are well represented in the sedimentary record. Most important among these are the diatoms, a phytoplankton group that is pervasive throughout the global ocean and often dominant in temperate to polar waters. The ocean's input/output budget of dissolved Si is likely important for variations in the characteristics and spatial variation of ocean productivity over Earth history. However, the longer-term dynamics of the ocean's Si reservoir and its impacts is too uncertain to safely summarize here. Instead, we focus on the dynamics of this nutrient in the modern ocean, which gives some insight into its possible changes through time.
Si is available in the same regions where N and P supply is rapid (e.g., in coastal upwellings and around Antarctica). However, Si is typically depleted "before" N and P; for example, in the Subantarctic Zone of the Southern Ocean, N and P are at relatively high concentrations in the euphotic zone, but Si concentrations frequently fall below the detection limit of standard analysis methods. While Si is consumed in the construction of diatom shells ("frustules"), the sinking and subsequent dissolution of frustules returns the Si to deep waters, analogous to the uptake/sinking/remineralization cycle of the nutrients held within organic matter. However, in comparison to N and P, it appears that biogenic opal on average sinks to greater depth. A substantial fraction (~25% on average) reaches the abyssal seabed before it is dissolved (Treguer et al. 1995), and a significant fraction of this is buried. Indeed, below the Southern Ocean, sediments can be nearly completely composed of biogenic opal. The "deeper remineralization" of biogenic opal probably contributes to the generally greater scarcity of Si relative to N and P in surface and shallow subsurface waters. Regional variation in the Si-to-N and Si-to-P ratio of diatom biomass also appears important in maintaining this Si scarcity (Sarmiento et al. 2004).
While Si availability is much lower in the tropical and subtropical ocean than in polar and temperate waters, diatoms are still present in these regions, where they are common in traditional microscopic examinations. It appears that the pervasive occurrence of diatoms even in the low latitudes is at least partly due to lower Si requirements (thinner frustules) in low latitude species and the vigorous recycling of Si within warm low latitude surface waters, where biogenic opal is more soluble. Despite such upper ocean Si recycling, diatoms are thought to contribute more to the sinking flux of organic matter (i.e., NEP) than they do to NPP (Buesseler 1998). At the simplest level, this may be due to their large size and opal frustules, which protect them from small zooplankton and help them to sink. In correspondence with their role in export production, diatoms appear to specialize on new nutrients imported from below, rather than nutrients recycled in the surface ocean.
In the Following Section: How Does Ocean Productivity Affect Atmospheric Carbon Dioxide?